3D printing in MRI and its application in an MRI compatible ex vivo beating heart

In this editorial, Andrew D Scott PhD (The Royal Brompton Hospital and Imperial College London; both London, UK) gives a sneak peek into research presented at 3DMedLIVE 2019: 3D printing in surgery, involving the use of 3D printing to design a chamber compatible for MRI scanning an ex vivo beating heart.

Biography
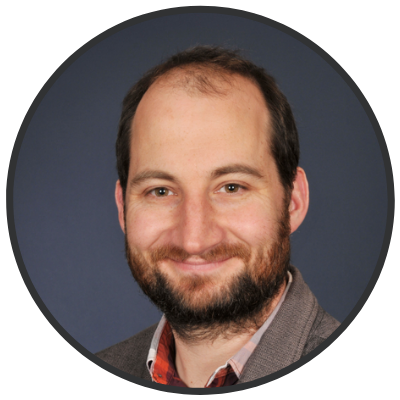
Andrew D Scott PhD is a Senior Physicist at the Royal Brompton and Harefield NHS Trust (London, UK), Honorary Senior Research Fellow at the National Heart and Lung Institute and a Research Fellow of the Data Science Institute, both at Imperial College London (UK). His role is research focused and he leads several independent projects.
Andrew is currently focused on understanding the links between measures obtained using diffusion tensor MRI methods and the underlying cardiac microstructure and making the imaging methods used in cardiac diffusion tensor imaging precise, reliable, robust and clinically relevant. He has an international reputation for cardiovascular MRI physics, in particular imaging the microstructure of the in vivo heart using diffusion tensor methods. In 2011 his contributions to cardiac MRI were recognised by being named a Junior Fellow of the International Society for Magnetic Resonance in Medicine and in 2018, a Fellow of the Society for Cardiovascular Magnetic Resonance.
Prior to taking up his current post in 2012, Andrew developed MRI techniques for the dynamic imaging of speech in pediatric patients with cleft palates and before that, completed his PhD in MRI coronary vessel wall imaging and respiratory motion correction at the Royal Brompton Hospital and Imperial College London.
>> Find out more about 3DMedLIVE
Register as a member of 3DMedNet for exclusive 3DMedLIVE updates
Magnetic Resonance Imaging (MRI) is a medical imaging technique that makes use of radiowaves and both a strong static and varying magnetic fields to produce images of the hydrogen nuclei within the body. Within a magnetic field, the hydrogen nuclei will absorb the radiofrequency energy and re-emit signals that can be detected. Images, therefore, show the distribution of water and fat and unlike x-ray based methods (including CT) and radiotracer based methods (SPECT and PET), an MRI scan does not have an associated ionizing radiation dose. The sensitivity to water and fat makes MRI well-suited to soft-tissue imaging and there are a number of contrast mechanisms available. These different acquisition strategies allow images to be produced with bright or dark blood, fat, muscle, white matter, grey matter and cerebrospinal fluid amongst other things. Many of these methods are based on intrinsic properties of the tissue that determine how quickly the intensity of the emitted radiowaves decays (time constant T2) and the subsequent recovery so that the experiment can be repeated again (time constant T1). Injection of chelated gadolinium contrast agent shortens T1 and allows tracer kinetic studies for assessment of perfusion, myocardial scarring, blood brain barrier permeability, tumor characteristics amongst other things. Advanced MRI methods provide quantitative measures of blood flow, the diffusion of water molecules and even the chemical composition of the tissue via spectroscopy.
While spatial resolution may be limited relative to CT and the method is more time consuming and expensive than ultrasound, the relative safety and flexible soft tissue contrast means that MRI is used clinically in a wide range of specialties and there is a highly active academic research community.
The excellent soft tissue contrast means that MRI images are often used as the basis for 3D printed models for surgical planning, patient information and training [1, 2]. One key area where 3D printing is likely to make substantial impact is in congenital heart disease. MRI based models of one or both of the blood pool and the heart and great vessels may be used in planning surgery or interventions or in aiding patients in understanding their condition [3].
One area where 3D printing is becoming important is in the construction of so-called phantoms, which are objects imaged with the MRI scanner (or other imaging modality) to evaluate the performance of the technology [4]. Phantoms are routinely used in the continuous quality assurance testing of medical imaging equipment and also in evaluating the performance of new techniques. Phantoms are often used to assess the signal to noise ratio (SNR) of a scanner, spatial resolution or the influence of various characteristics of the imaged tissue on the images (for example motion). Construction of ideal phantoms can be expensive and in some cases, difficult or even impossible. 3D printing allows the cost-effective production of one-off phantoms or reproducible production of multiples. Most commonly, a 3D printed shell is created and filled with oil and/or water based materials. 3D printed regions can be used to produce signal voids when producing phantoms, for example, for assessing resolution or geometric distortion [5]. In this type of phantom, prints must be constructed without material saving reduced fill-factors as the air-filled cavities result in image artefacts due to sharp changes in the magnetic susceptibility at the air-plastic interface. Fused deposition modelling prints can also be problematic due to the ingress of water or oil into the print, which would result in partial signal from the regions designed to be signal free. Coating the surface with an impermeable spray may solve this problem (https://phantoms.martinos.org/ ) or the water may be allowed to penetrate the filament gaps in order to provide microscopic structure for the assessment of diffusion-based MRI techniques [6].
Another class of 3D printed phantoms are anatomically realistic phantoms based on scans of patients. These phantoms can be patient-specific and have been used to validate computational fluid dynamics simulations of flow through complex vasculature with validation using MRI-based flow measurements [7]. 3D printed whole head phantoms based on actual MRI data have found use in measuring the MRI related tissue heating [8] and the effects of magnetic field changes due to air filled cavities within the head [9]. The majority of phantoms used in MRI have been directly printed and then filled with MRI visible fluids, while for application in ultrasound and x-ray computed tomography (CT), molds are often printed and then used to produce the tissue-replicating structure that is itself imaged, as they do not rely on the hydrogen nuclei for imaging signal [4]. However, some 3D printed materials have been imaged directly with MRI, although the T1 and T2 properties of the materials were rather unrealistic for most tissues of interest in the body [10].
In pre-clinical MRI research several groups have 3D printed a mold of the left ventricular cavity of the heart of pigs based on images acquired in the beating in vivo heart [11][12]. This mold can then be placed into the excised heart to minimize the deformation and contraction that otherwise results in substantial differences between the in vivo and ex vivo heart. These filled, ex vivo hearts can then be imaged over many hours or even days at spatial resolutions that would be impossible in the beating heart.

At 3DMedLIVE 2019, I will present our recent pre-clinical work in developing an MRI compatible large animal ex vivo beating heart setup using 3D printed equipment. The aim of the work was to assess the effects of the heart motion and strain on MRI based measures of the microscopic structure of the heart. Our collaborators in Bristol (UK) removed hearts from pigs based on a heart transplant protocol. These arrested hearts were transported by motorcycle courier to the Royal Brompton Hospital (London, UK), where we connected the hearts to a modified heart bypass circuit. Using a method named after its inventor, Oskar Langendorff, by pumping a warm solution containing salts, glucose and oxygen down the aorta, the aortic valve is pushed closed and this perfusate is forced down the coronary arteries (Figure 1) feeding the heart muscle [13] and allowing it to start beating again (Figure 2). We scanned the beating heart and then switched the perfusing solution for a formulation that stopped the heart in a relaxed state and then again switched to a solution that caused contraction.
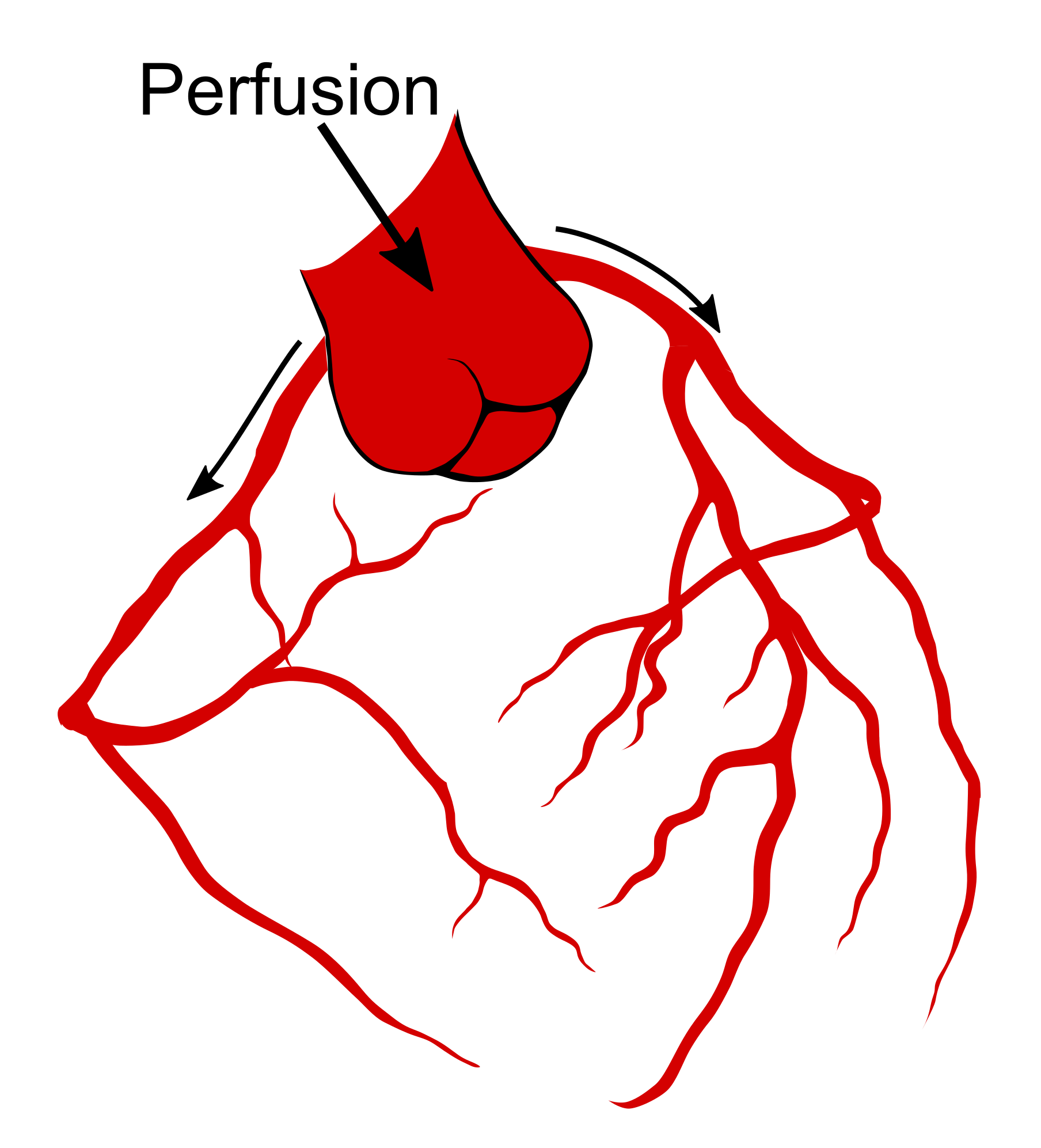
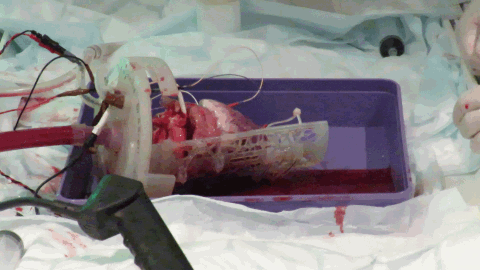
While off-the-shelf solutions exist for Langendorff perfusion of hearts, they rely on many metallic parts. The large magnetic field constantly produced by the MRI scanner means that these commercially available Langendorff systems are MRI-unsafe due to the risk of iron-based parts becoming projectiles. We worked with 3D printing expert, Alex Berry to design a chamber to hold the beating Langendorff pig heart at the center of the MRI scanner (see Figure 3). The aortic cannula is integrated into the chamber lid, along with a number of ports allowing pressure, temperature, ECG and pacing connections to enter the chamber. The heart on the cannula is supported by a Voronoi patterned tray, which has oil filled tubing snaked along the bottom face. The tubing is visible on the MRI scans and allows co-localization of the imaging slice with a section of tissue removed for subsequent histology. The lid twists to seal onto the face of the chamber itself using a dual o-ring seal within the front face. Connectors on the side and bottom of the cylindrical chamber allow it to be used with either the heart lying on its side, supported by the tray or hanging vertically from the aortic cannula.
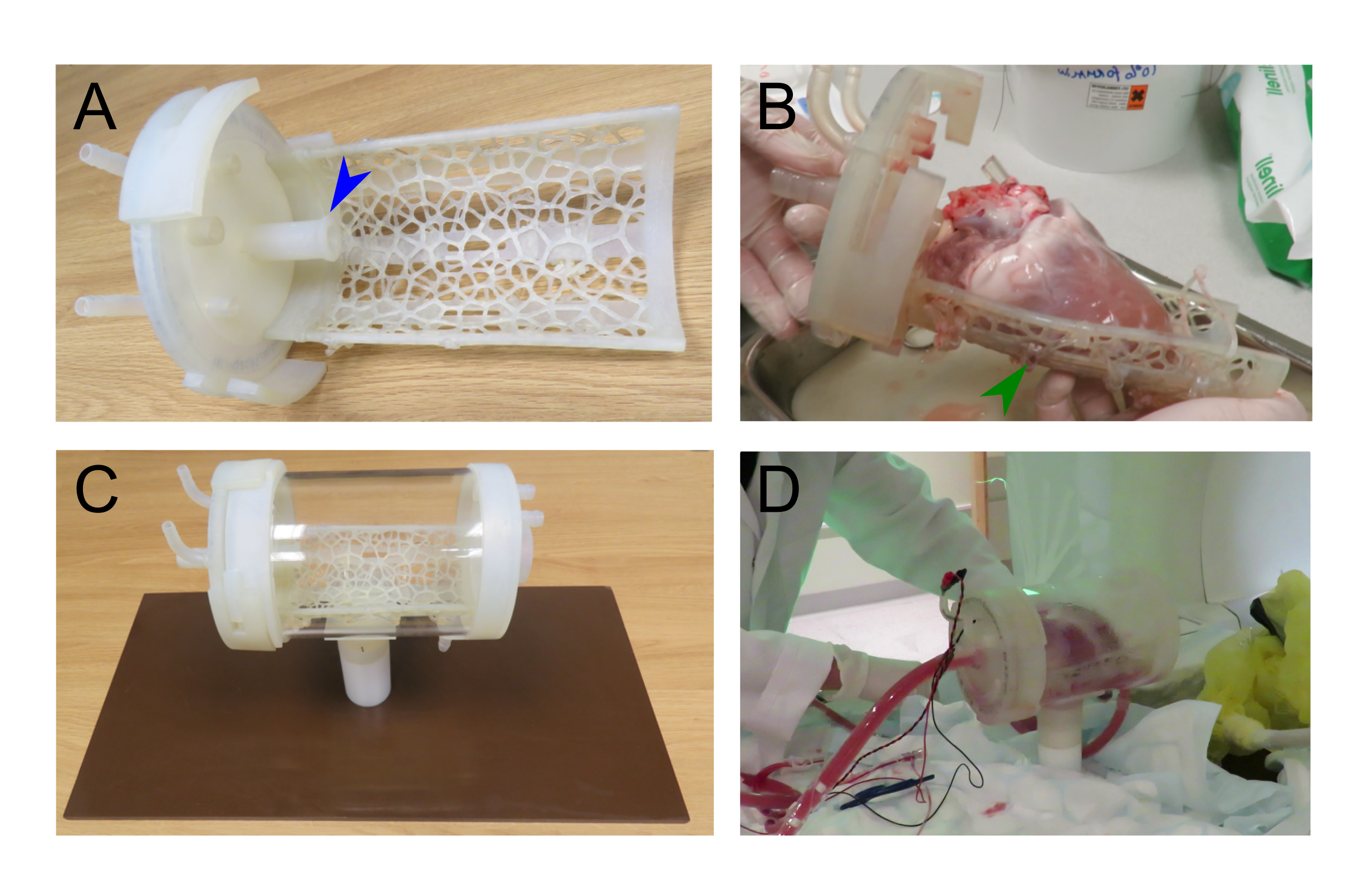
Apart from the cylindrical acrylic tube, the phenolic sheet base and PTFE upright support, all parts were 3D printed using a stereolithographic system (Form 2, Formlabs; MA, USA) in a semi-flexible material (Durable resin), including plugs for the drainage ports to seal the chamber. By 3D printing the parts of the chamber, we were able to produce smaller prototype chambers and produce and test a number of MRI compatible chamber designs.
The use of a SLA printer meant that a sufficiently competent seal could be achieved with an o-ring and machine-milled ring fixed into the 3D-printed lid.
MRI and 3D printing are compatible technologies. The highly active MRI research community is keen to utilize the rapidly produced, non-metallic parts available with 3D printing and it is likely that the number of MRI research publications making use of 3D printing will rise. Bioprinting may one day enable increasingly more realistic phantoms with tissue-like MRI properties and the use of 3D printing in producing custom parts for pre-clinical studies will soon be the norm.
- Colleagues at the Cardiovascular Magnetic Resonance Unit, Royal Brompton and Harefield NHS Foundation Trust and Imperial College London. (all London, UK)
- Professor Raimondo Ascione and Team at the Translational Biomedical Research Centre, University of Bristol (UK)
- Alex Berry (UK)
- Tim Jackson and Team, Perfusion, The Royal Brompton and Harefield NHS Foundation Trust
- Robin Hardie, Clinical Engineering, The Royal Brompton and Harefield NHS Foundation Trust
[1] Giannopoulos AA, Mitsouras D, Yoo SJ, Liu PP, Chatzizisis YS, Rybicki FJ. Applications of 3D printing in cardiovascular diseases. Nat. Rev. Cardiol. 13, 701—718 (2016).
[2] Parthasarathy J, Krishnamurthy R, Ostendorf A, Shinoka T, Krishnamurthy R. 3D printing with MRI in pediatric applications. J. Magn. Reson. Imaging. doi: 10.1002/jmri.26870 (Epub ahead of print) (2019).
[3] Biglino G, Koniordou D, Gasparini M et al. Piloting the use of patient-specific cardiac models as a novel tool to facilitate communication during clinical consultations. Pediatr. Cardiol. 38(4), 813—818 (2017).
[4] Filippou V, Tsoumpas C. Recent advances on the development of phantoms using 3D printing for imaging with CT, MRI, PET, SPECT and ultrasound. Med. Phys. 45(9), 740—760 (2018).
[5] Bieniosek MF, Lee BJ, Levin CS. Technical note: characterization of custom 3D printed multimodality imaging phantoms. Med. Phys. 42(10), 5913—5918 (2015).
[6] Abu-Sardanah SO, Baron CA, Moore J, Peters T, Khan AR, Hussain U. Design and evaluation of a diffusion MRI fibre phantom using 3D printing. Proc. SPIE 10573, Medical Imaging 2018: Physics of Medical Imaging, 105731Q (2018).
[7] Anderson JR, Diaz O, Klucznik R et al. Validation of computational fluid dynamics methods with anatomically exact, 3D printed MRI phantoms and 4D pcMRI. Conf Proc IEEE Eng Med Biol Soc. 2014. 6699—701 (2014).
[8] Graedel NN, Polimeni JR, Guerin B, Gagoski B, Bonmassar G, Wald LL. An anatomically realistic temperature phantom for radiofrequency heating measurements. Magn. Reson. Med. 73(1), 422—450 (2015).
[9] Guérin B, Stockmann JP, Baboli M, Torrado-Carvajal A, Stenger AV, Wald LL. Robust time-shifted spoke pulse design in the presence of large B0 variations with simultaneous reduction of through-plane dephasing, B1+ effects, and the specific absorption rate using parallel transmission. Magn. Reson. Med. 76(2), 540—554 (2016).
[10] Rai R, Wang YF, Manton D, Dong B, Deshpande S, Liney GP. Development of multi-purpose 3D printed phantoms for MRI. Phys. Med. Biol. 64(7), 075010 (2019).
[11] Roujol S, Tschabrunn C, Basha TA, Kissinger KV, Manning WJ, Josephson ME, Anter E, Nezafat R. A novel framework for unified analysis of in vivo and ex vivo cardiac data using an in vivo MRI-derived 3D printed model: application to cardiac MRI. Proc. Int. Soc. Magn. Reson. Med. Annu. Meet. 2584 (2015).
[12] Cork TE, Perotti LE, Verzhbinsky IA, Loecher M, Ennis DB. High-resolution ex vivo microstructural MRI after restoring ventricular geometry via 3D printing. Proc. Funct. Imaging Model. Hear. – Lect. Notes Comput. Sci. 177—186 (2019).
[13] Zimmer HG. The Isolated Perfused Heart and Its Pioneers. Physiology. 13(4), 203—210 (1998).