Three key advanced scaffold fabrication methods for tissue engineering
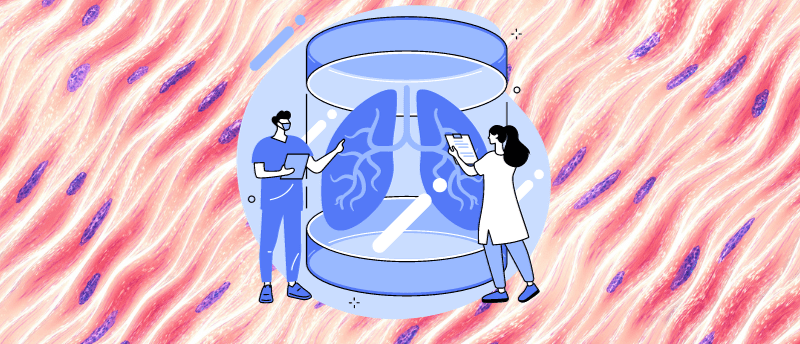
One of the primary goals of tissue engineering is to produce functional tissue constructs that may be used to overcome dysfunctional tissues and organs [1]. Scaffolds are one of the key components of functional tissue constructs, alongside cells and cellular signals [5]. Here, we present three advanced scaffold fabrication methods that enable the generation of more intricate tissue constructs.
Three-dimensional printing
3D printing encompasses a range of techniques that create scaffolds in a layer-by-layer manner [2]. In the context of tissue engineering, such techniques produce complex tissue constructs that mimic native organs and tissues [3]. Methods like selective laser sintering or stereolithography are capable of producing highly porous, interconnected and patient-customizable scaffolds [6]. These have been improved upon with extrusion and inkjet printing, which allow for scaffolds with higher resolution and the ability to embed drugs, cells, growth factors or signaling and cellular interaction molecules. This allows for the more accurate replication of native tissues and their bioactivity [1,6].
In addition to the actual printing technology employed, two methodological approaches can be considered: scaffold-based or scaffold-free [4]. In scaffold-based bioprinting, an exogenous biomaterial matrix is produced, which is then seeded with cells or has cells embedded as part of a cell-laden bioink. Scaffold-free printing, on the other hand, relies on the fusing of cellular aggregates to form tissue constructs. Scaffold-free approaches are effective in producing small tissue scaffolds with high cell densities, while scaffold-based approaches are more apt for producing large, complex structures that require mechanical robustness e.g., bone tissue [4].
Dermal, neural, cardiac, musculoskeletal and tracheal tissue constructs have been successfully produced from 3D-printed scaffolds [1]. While attempts are underway to produce large, complex organs such as the human heart [10], a significant challenge remains evident: it is vastly difficult to replicate the vascularization inherent in large organs [5]. In its current state, bioprinting often involves creating small-sized scaffolds and tissue constructs, underscoring these limitations.
Electrospinning
Electrospinning is a tissue construct production method that fabricates microfibre-forming scaffolds. This occurs by passing an electrically charged polymer solution through a spinneret, a needle with a metal tip, and onto a collecting plate. The process is driven by the potential difference in charge between the spinneret and the collecting plate, which draws the polymer solution into a microfibre through electrostatic forces [4]. This technique allows for the generation of highly porous and interconnected scaffolds with large surface areas [7].
Several modified versions of electrospinning contribute to even more complex microstructures and subsequently advanced scaffolds, such as coaxial or triaxial electrospinning, which enable the formation of multiple-layered fibers. These can be designed to consist of different polymers and also be used to deliver therapeutic molecules [1], thus increasing the intricacy of the tissue construct.
Other modifications include cell-laden electrospinning, which produces cell-embedded fibers, and melt electrospinning, which is capable of producing nanofibers instead of microfibres [1].
Electrospinning has been successfully employed in the generation of scaffolds for functional bone, cartilage, neural, dermal and blood vessel constructs [1]. However, it is limited to the production of fibrous scaffolds and consequently, can only produce models for certain tissue types. In addition, electrospinning provides less control over the pore size and shape within 3D scaffolds when compared to other methods of assembly [6].
Decellularized tissues
Decellularization is a process that extracts an autologous, allogeneic or xenogeneic extracellular matrix (ECM) scaffold from organs, tissues or cells [9]. This leaves a decellularized extracellular matrix (dECM) with structural and biochemical cues intact, yet devoid of native cells or genetic materials [8]. The retention of such cues enables the improved reseeding of the dECM with new cells.
The source of dECM falls into two large categories: organ/tissue-derived or cell-derived [9]. The former are larger scaffolds produced from specific organ or tissue types, which retain their natural 3D architecture but remove immunogenic cellular components, while the latter is harnessed from the secreted ECM of in vitro cells that are then decellularized. Both have distinct advantages: cell-derived dECMs are comparatively easy to produce and have a lower risk of adverse host immune responses than whole organ or tissue dECM. Meanwhile, organ and tissue-derived dECM retain memory factors, architectures and physiochemical cues that confer a tissue-specific memory, which efficiently drives and regulates reseeded cell adhesion, proliferation and differentiation [9], making the resulting tissue construct more representative of and synergistic with native tissue.
The methods of decellularization include physical or enzyme- and chemical-based techniques, which either immerse tissues in cell lysing agents or circulate these agents through their intrinsic vasculature, thereby removing cellular contents [9]. Enzyme-based approaches offer a higher specificity for specific cells and features nucleases, trypsins, lipases and phospholipases. Chemical-based methods utilize ionic and non-ionic detergents or chemical agents such as acids, bases, hypertonic, hypotonic and chelating agents. Physical decellularization approaches include but are not limited to freeze-thawing, sonication and electroporation of the tissue.
Owing to the specific size and cellular characteristics of tissues there is no standardized practice of decellularization [9]. Rather, different combinations of physical, chemical and enzyme-based approaches are employed to maximize bioactivity and cell seeding potential of the specific tissue type [1]. For instance, a 2018 study from Rahman et al optimized the decellularization of auricular cartilage, the connective tissue often referred to as gristle, using a cell-lysing combination of sodium deoxycholate detergent and enzymatic agents, specifically trypsin and deoxyribonuclease. Additionally, a crucial step in this process involved freeze-thawing, implemented to minimize the exposure to cell-lysing in order to retain crucial biochemical and biomechanical properties of the scaffold [10].
Decellularized scaffolds are more effective in replicating native tissues than synthetic scaffolds, but they face the risk of scaffold rejection through residual cellular or genetic contents. If done ineffectively, decellularized scaffolds can result in immunorejection [8], which is of a higher risk for allogeneic or xenogeneic-derived scaffolds. This can result in severe medical complications like graft-versus-host disease, rendering the tissue construct more harmful than beneficial in clinical settings.
Combinatorial scaffold fabrication methods
Such advanced scaffold fabrication methods can generate scaffolds on their own, but they can also be used in concert to generate specific and complex types of scaffolds and tissue constructs.
Electrospinning can be combined with 3D printing by collecting the micro- or nanofibers and using these deposited fibers as the material being deposited by the 3D printer [1]. This enables finer control of fiber deposition and also the enhancement of the scaffold’s mechanical properties. Furthermore, it can also improve cell proliferation and infiltration of the scaffold by seeding cells.
Decellularized scaffolds can also be combined with other methods. This occurs by generating a decellularized scaffold through the aforementioned techniques and then solubilizing it into a biomaterial, that is either 3D printed [9] or electrospun [12] into a scaffold. These have been used mainly in the generation of cartilage, neural, adipose, muscle, hepatic, bone, dermal and lung tissue constructs. However, a number of tissue-specific limitations, like long-term viability of cardiac tissue or load-bearing qualities of cartilage tissues, need to be overcome to see the translation of these promising technologies into clinical technologies [13].
The technologies described in this article have already yielded a variety of tissues, ranging from cardiac to bone, but to progress this technology toward clinical translation there are some pivotal challenges to overcome. Namely the improvement of tissue construct viability through enhanced vascularisation and angiogenesis or altered fabrication methods to integrate tissue-specific properties. With these technologies under constant development, however, hopes for a future where tissue engineering can produce readily accepted tissue constructs for a host of regenerative applications remain bright.
This article is part of our Spotlight on tissue engineering
Visit the Spotlight, produced in association with Cook MyoSite, to delve into the current landscape. It covers the breakthroughs, challenges, and exciting applications that are unfolding in the field.