CRISPR: taking the short-cut to a healthy genome editing enterprise
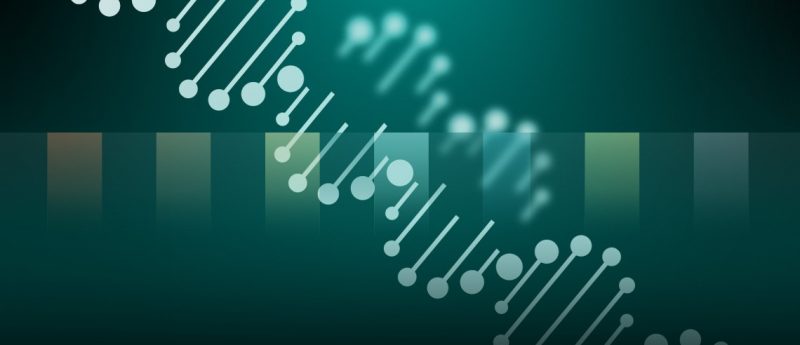
In under 3 years, CRISPR—Cas9 has seen unprecedented rise in funding, patents and publications, with first ideas already progressing to the clinic. For a field that’s poised to deliver, can we envision the translational, policy and business paths for a long-term success?
Methods and tools for ‘genetic engineering’ or ‘genetic modification’ have been used successfully for more than 3 decades in animal models, as well as treated a number of patients in early gene therapy trials. Until recently, specificity challenges and downstream laborious processes have been upping the stakes in curing genetic disease. However, a number of scientific breakthroughs have led researchers to build tools that edit genomes with unprecedented specificity, efficiency and precision, enabling the modification of any part of the genome [1].
The use of precision genome modification has exploded, swelling hopes for the elucidation, modelling and treatment of disease, also creating a surge of companies looking to build and capitalize on the technologies as research tools, novel approaches in agriculture and transformative treatments. In light of growing global excitement and ongoing scientific success in countries like the US and UK, there is a timeliness and urgency to support the evolution of a sustainable gene editing field and deliver its promise for a clear economic and social benefit. Building off of this momentum and drawing upon a rapidly growing body of literature and public forums, this report aims to identify critical parameters impacting the acceleration of genome editing technologies, particularly the CRISPR—Cas9 system, and the development of transformative tools and products.
The last cut is the deepest?
Compared to traditional genetic engineering methods, the nascent gene editing tools using zinc finger nuclease (ZFN), transcription activator-like effector nucleases (TALENS) and clustered regularly interspaced short palindromic repeats (CRISPR), have allowed genome studies with increased efficiency [2]. All three engineered systems essentially act as molecular scissors inducing double strand DNA breaks, thus allowing the knock-out, replacement, mutation or correction of specified regions.
The CRISPR—Cas9 system in particular, the last of the breakthroughs to emerge in 2012, is frequently paralleled to the polymerase chain reaction (PCR) in its disruptive potential [3]. In the hands of bioengineers CRISPR was turned from a bacterial immune system into a molecular ‘Swiss-knife’ that can be used in any cell type to target any gene of interest [4]. The system deploys the enzyme Cas9 to cut the DNA at genomic sites specified through an engineered RNA guide sequence. The cuts are then repaired by the cell’s natural DNA-repair processes. This DNA/RNA-guided genomic regulation makes CRISPR—Cas9 simpler to use compared to the other two DNA-binding protein based systems.
A number of recent technology modifications have further propagated its scientific and clinical potential. These include novel platforms based on a nuclease-deactivated Cas9 (termed dCas9) that can mediate both transcriptional repression through CRISPR interference (CRISPRi) and CRISPR activation (CRISPRa) [5]. The development of dCas9 can potentially augment the system’s versatility for disease research, enabling novel platforms that control gene expression without genomic alterations. Examples include the capacity for transient, stable, or quantitative gene regulation, as well as the concomitant manipulation of multiple-genes.
Although its efficiency and simplicity has turned genome editing into standard practice, the remarkable precision, versatility and ease of CRISPR—Cas9 has been gradually dethroning its predecessors, making it the system of choice in the majority of basic and clinical research projects [6].
Editing solutions
There are thousands of human genetic diseases. In the past 30 years, the vision to provide life-long curative treatments by targeting their root cause, was met by traditional gene therapy approaches to replace or silence faulty genes, which, however, leave the underlying gene sequences intact. The nascent targeted nuclease systems enable the permanent molecular correction of pathogenic gene mutations, setting the ground for a whole new generation of putatively transformative therapeutics. The demonstration that all three technologies can target endogenous genes in human induced pluripotent stem cells, marked a major watershed in this respect [7].
Although conventional gene therapy and genome editing share a large pool of target diseases, the latter can readily offer a solution when disease genes are too large for vector systems or their replacement is not enough for a therapeutic outcome. Genome editing has already shown encouraging results in models of haematological diseases, immune deficiencies and cancer, as well as the clinic with the use of ZFNs against HIV [8].
There is conserved enthusiasm that genome editing can eventually be deployed to target all levels of molecular disease complexity, affecting any organ in the body. Conceivably, the ‘low-hanging’ fruits include monogenetic conditions such as thalassemias or anemias, diseases requiring partial cell correction for benefit, as is severe combined immunodeficiency (SCID), as well as easily accessible organs, like the eye and the liver. However, its true potential is shaped by a number of key factors, including a monogenic or polygenic disease aetiology, the fraction of cells that have to be corrected and the type of necessary modifications, as deleting or replacing genes can be easier than yielding precise corrections. Not unlike traditional gene therapy, the delivery of editing systems to the right cells or organs remains an important challenge and object of intense research.
The race of genome editing tools to the clinic is nothing sort of excitement and the relative efficiencies of TALENs, ZFNs and the CRISPR—Cas9 are already being put to the test. Due to its higher precision and ease of use, in a very small window of time CRISPR has made an impressive leap from research tool to a promising therapeutic platform [9], already showing encouraging results in preclinical models of haematopoiesis in sickle-cell disease [10] and HIV [11], as well as T-cells [12]. CRISPR’s promise to revolutionize health care does not stop to single disease treatment. Recent reports on the simultaneous modification of more than 60 DNA sites in pig embryos might have brought us closer to the generation of non-human transplantable organs [13].
‘Cutting’ to the chase: Accelerating global market potential
Although ZFNs, the first editing tools, go back more than a decade, it took the advent of CRISPR to generate the kind of market pull seen with breakthroughs like RNAi and monoclonal antibodies. From the establishment of the non-profit organization Addgene that distributes CRISPR plasmids to the research community, to the recent launch of eGenesisBio in Boston for the production of the genome modified organ-donor pigs, companies are in an arms race to deliver the therapeutic promise of CRISPR—Cas9.
With a constellation of global investors already lining up, including the Gates Foundation and Google Ventures, companies targeting the development of CRISPR—Cas9 clinical approaches and products have raised hundreds of millions in record time [14]. Companies like Editas Medicine, CRISPR Therapeutics, Caribou Biosciences and Intellia Therapeutics are among the pioneers of the CRISPR technology, with Sangamo BioSciences currently the only focusing on ZFNs [15]. Recent multimillion partnerships and acquisitions from established gene therapy pioneers pay further dividends to the technologies’ significant potential. Notable examples include the partnership of Juno Therapeutics with Editas on the development of novel genome-edited immunotherapies [16], the collaboration of Switzerland-based CRISPR Therapeutics with US Vertex Pharmaceuticals [17], as well as the acquisition of Precision Genome Engineering by Bluebirdbio [18].
Crossing the pond
Alongside the inarguable leading position of the US, the UK has been one of the first to acknowledge the vast potential of genome editing. Multidisciplinary research across its world leading academic institutes, including the Wellcome Trust Sanger, the Francis Crick Institute and several Universities and MRC centres, is steadily establishing the foundations to enable the UK maximize its global scientific contributions, respond to new developments and market opportunities and grow a new enterprise. Signs of the latter have already started to show with start-ups like Desktop Genetics, established biotechs such as Horizon Discovery and global pharma giants like AZ already capitalizing on the technology to develop enabling tools and platforms.
Despite its early stage, the volume of ongoing work to link basic genome editing science with promising applications underscores its considerable potential to generate both underpinning tools and products. However, the majority of nascent companies and ventures do not possess the scientific or financial capacity to deliver a novel technology to commercialization within the current, punishing investment windows. Building upon the growing scientific foundations, strategic and long-term commitment is needed to unlock the commercial and business potential of new genome editing technologies.
Editing out barriers
Moving research and applications towards the market calls for a clear understanding of the challenges and opportunities that define the boundaries between promise and reality. As in many other breakthrough areas, an important step in this direction involves the early engagement and collaboration of the research and business communities with regulatory and government authorities, on the development of standards and guidelines for safety and efficacy assessment.
1. Aim-Shoot-Edit:
Gene editing technologies, and particularly the CRISPR/ Cas9 system, are still very early in the innovation lifecycle, requiring higher levels of scientific consistency and integrity. Although the promise of clinical applications has been put in the spotlight, these technologies continue to hold unprecedented value as tools for basic research. Distinct topics of scientific inquiry include the progression of genome editing science per se, the development of editing tools for the elucidation of biology and disease and proof of concept research on their therapeutic potential.
Efficiency: The vast differences between editing systems offer solid proof that we have barely scratched the surface [19]. The two older editing technologies pale beside the gene correcting efficiency of CRISPR, yet the latter possesses significant limitations when size is important. Packaging of both CRISPR and TALENs into adeno-associated viral vectors (AAV), system of choice for numerous gene therapies, is often problematic. ZFNs have a distinct advantage with vector systems, although the discovery of a shorter Cas9 gene from Staphylococcus aureus, has already allowed AAV-mediated use of CRISPR in animal liver cells [20], [21]. The team of Feng Zhang, one of the CRISPR pioneers, also discovered a new CRISPR protein, Cpf1 that demonstrates several functional advantages to Cas9, thus opening the door to new treatments [22].
Control: A particular concern with genome editing tools are unintended cuts at similar or identical DNA sequences that appear in different parts of the genome (off-target sites). These could raise major safety concerns, were the technologies to progress to the clinic. The frequency of off-target effects varies among the different systems, with TALENs showing increased stability due to their longer target DNA sequences [23]. Efforts to increase the specificity of CRISPR—Cas9 include adjusting the guide RNA, tweaks in the structure of Cas9 to regulate its lifespan and varying the enzyme concentrations.
Boost: These results are a continuous proof of the long and winding road ahead, as well as the many lessons and solutions that scientists can decipher from a growing understanding of the evolutionary biology of natural CRISPR systems. Most importantly, they highlight the importance of efforts ahead of any clinical application, to minimise the impact of genome-wide off-target binding, improve viral vector and alternative delivery systems for gene therapy and control the extent of editing capacity after initial target modification.
2. From double strands to value chains
As is usually the case with breakthrough innovations, investment enthusiasm often comes with unrealistic timelines for commercialization. The tenacious strides and challenging commercial successes of Chondrocelect [24] and Glybera [25], market pioneers in regenerative medicine and gene therapy respectively, speak volumes of the winding road ahead of genome editing solutions. With cell and gene therapies finally coming of age after 3 bumpy decades, there is an abundance of learnings to be mined while genome editing applications are still some way from the clinic.
Modifying Policy: When it comes to regulation, high quality standards are a necessary, yet not adequate condition in ensuring that patients can benefit from innovative approaches and products. From gene therapy to dendritic vaccines and immunotherapies, regulatory bodies have been grappling with the assessment of one-off, life-long, curative approaches and the capacity to predict long-term value for patients, as well as health systems [26].
In an effort to absorb emerging breakthroughs, global health systems have been reinventing themselves through a number of policy updates and reforms for the speedier and cost-effective development, evaluation and adoption of innovative medicines and technologies. Sourcing the experience from the 70+ regenerative medicine / cell therapy products and 5 gene therapies approved to date, the European Medicines Agency (EMA) has already issued guidelines to help innovators create a clear route to market for novel therapeutic approaches [27]. A number of mechanisms to speed up licencing are also in place across several jurisdictions, including the FDA’s Breakthrough Therapy designation, Fast Track designation and Priority Review Pathway, matched by the EMA’s Accelerated Assessment program in Europe [28].
Cutting back on time and risks: Yet, as the rich history of cell and gene therapies teach us, licencing is far from the journey’s end. Alongside a number of clinical setbacks, the lack of a clear expertise, decision making requirements and a pathway to achieve clinical and commercial success has significantly delayed the delivery of many potentially transformative solutions. Contrary to established thinking, business success is more of a continuum than single-gated approval process, involving decisions across all stakeholders; from researches and manufacturers, to regulators, payers, clinicians and patients. The continuous cycle of evidence on safety, real-world effectiveness and economic value that are required to assess medical innovations, suggest that product development, licensing, coverage and adoption interlink and could indeed occur in parallel.
Crossing stakeholder boundaries: Particularly in areas like genome editing, scientific knowledge growth unarguably exceeds the pace of policy change, putting regulation to the test. The unprecedented progress seen with CRISR systems, underscores the need for flexible and adaptive regulatory frameworks to capture and respond to emerging information and the inherent uncertainty of innovation [29]. Moving beyond the established boundaries, in 2014 the EMA launched the Adaptive Pathways pilot as a new program that spans across product development to support an earlier and progressive patient access through greater stakeholder engagement, dialogue and iterative reviews [30]. Stakeholder engagement efforts are already underway, such as the Innovative Medicines Initiative (IMI2) ADAPT-SMART program. These involve input and dialogue among players at different stages across product development, not least the patients, to align priorities, clarify evidence requirements and achieve the trade-offs necessary to accelerate the timely and cost-effective adoption of transformative treatments. The breakneck progression of Sangamo’s ZFN approach to the clinic has been in part attributed to such a stronger patient engagement, further adding a new page to the well-trodden activism history of AIDS patients [31].
In the case of genome editing, other burning issues make multi-stakeholder dialogue indispensable. Recent reports that CRISPR—Cas9 has been used in non-viable human embryos to modify the gene responsible for β-thalassaemia, has fuelled dystopian future scenarios on the modification of the human germline [32]. Any embryo genome modifications are heritable to the next generations, kicking the hornet’s nest of safety, moral and legal concerns over the broader use of these technologies [33]. In light of constant scientific developments, it will be key to open the dialogue between governments, scientists, as well as the public and device new inclusive frameworks for scientific regulation that can capture unanticipated risks, while allowing for advancements to progress. Conceivably, there’s a clear distinction between the somatic therapeutic application of editing tools, where investors and companies are now cramming and their unregulated reproductive use, which will be critical to include in ongoing legislative reviews and public deliberations [34].
Filling the nicks: building a robust and sustainable biomedical sector
For many, genome editing tools may revolutionize even the most transformative of medical practices, such as cell and gene therapies. Although a number of critical factors seem to hold the key to the field’s progression, addressing barriers in isolation is not enough. Within a rapidly changing scientific landscape, it is also important to identify core themes spanning from the elucidation of technological and translational bottlenecks, to updates in policy, regulation and investment models.
The UK in particular, albeit not the birthplace of genome editing, possesses a world-leading academic expertise, strong government commitment and a number of forward looking development and adoption frameworks to back both synthetic biology and the field of cell and gene therapies, as two of its 8-great technologies [35]. The latter include a number of reforms, such as the Early Access to Medicines Scheme of the Medicines and Healthcare Products Regulatory Agency (MHRA), new scientific advice and technology appraisal projects by the National Institute for Clinical Excellence (NICE), and the Accelerated Access Review initiative of to establish novel adoption pathways of innovation to the National Health System (NHS). Moreover, Innovate UK, the government’s organization to identify and promote promising science and technology innovations, opened its 2015 competition in Developing Regenerative Medicines and Cell Therapies to genome editing solutions, in an attempt to further motivate and stimulate the local ecosystem. In the race to build a fledgling industry, the establishment of system-wide infrastructures and investment in areas with unclear value propositions or business models, can put the UK in an advantageous position to embrace and propel disruptive technologies, in an otherwise uncertain global environment.
For a potentially disruptive, yet not-fully deciphered technology to fulfil its therapeutic and market potential, scientists, health systems, businesses and government must hold hands. They also need to take a holistic view of the innovation pathway: anticipate scientific and technical risks, facilitate maturation of applications, provide financial support mechanisms and promote a more connected and collaborative environment for innovation. One that can incorporate the views of a range of key stakeholders, both within the local ecosystems and globally.
//Disclaimer//
The views and opinions expressed in this article are those of the author and do not necessarily reflect the official policy or position of any current or former affiliations.
REFERENCES
- Petherick, A. Nature 528, S1 (2015), doi:10.1038/528S1a
- 2.Maxmen, A, Nature 528, S2—S3 (2015), doi:10.1038/528S2a
- Ledford, H. Nature 522, 20—24 (2015), doi:10.1038/522020a
- Jinek, M.et al. Science 337, 816—821 (2012).
- Dominquez, A.A. et al., Nature Reviews Molecular Cell Biology 17, 5—15 (2016) doi:10.1038/nrm.2015.2;
- Carroll, D. Annu Rev Biochem. 83, 409-39 (2014), doi: 10.1146/annurev-biochem-060713-035418
- Byrne et al., Nucl. Acids Res.(2014), doi: 10.1093/nar/gku1246
- Perez, E.E. et al., Nature Biotechnology 26, 808 – 816 (2008) | doi:10.1038/nbt1410
- 9. Sato, T and Clevers, H. Science 340, 1190-1194 (2013), DOI: 10.1126/science.1234852
- Canver, M.C. et al. Nature 527,192—197 (2015), doi:10.1038/nature15521
- Mandal, Pankaj K. et al. Cell Stem Cell 15, 643 — 652 (2014) DOI: http://dx.doi.org/10.1016/j.stem.2014.10.004
- Schumann, K. et al. PNAS112 (33), 10437-10442 (2015) doi:10.1073/pnas.1512503112
- http://www.nature.com/news/gene-editing-record-sma…
- http://www.bizjournals.com/boston/blog/bioflash/20…
- http://www.nanalyze.com/2015/04/7-gene-editing-com…
- http://www.bostonglobe.com/business/2015/05/27/med…
- http://www.european-biotechnology-news.com/news/ne…
- http://www.bluebirdbio.com/our-focus/gene-editing/
- Gaj, T. et al. Trends in Biotechnology 31 (7), 397 — 405 (2013) DOI: http://dx.doi.org/10.1016/j.tibtech.2013.04.004
- http://www.nature.com/news/mini-enzyme-moves-gene-…
- Ran, F. A. et al. Nature 520, 186—191 (2015) doi:10.1038/nature14299
- http://www.nature.com/news/alternative-crispr-syst…
- Corbyn,Z. Nature 528, S4—S5 (2015) doi:10.1038/528S4a
- Gerlier, L. et al. Pharmacoeconom. 28(12), 1129-46, (2010) doi: 10.2165/11584920-000000000-00000
- http://www.bloomberg.com/news/articles/2015-05-21/…
- Bianco, P..and Sipp, D. Nature 510, 336—337, (2014) doi:10.1038/510336a
- http://www.ema.europa.eu/ema/index.jsp?curl=pages/…
- Baird, L. et al. Clin. Pharmacol. & Therapeut. (2014); 96 5, 559—571. doi:10.1038/clpt.2014.145
- Oye, K. A.et al. Science 345, 626—628 (2014) DOI: 10.1126/science.1254287
- http://www.ema.europa.eu/ema/index.jsp?curl=pages/…
- Eisenstein, M. Nature 528, S8—S9 (2015) doi:10.1038/528S8a
- Liang, P. et al. Protein Cell 6, 363—372 (2015) doi: 10.1007/s13238-015-0153-5
- Lanphier, E. et al. Nature 519, 410—411 (2015) doi:10.1038/519410a
- http://www.nature.com/news/us-science-academies-ta…
- https://www.gov.uk/government/publications/eight-g…